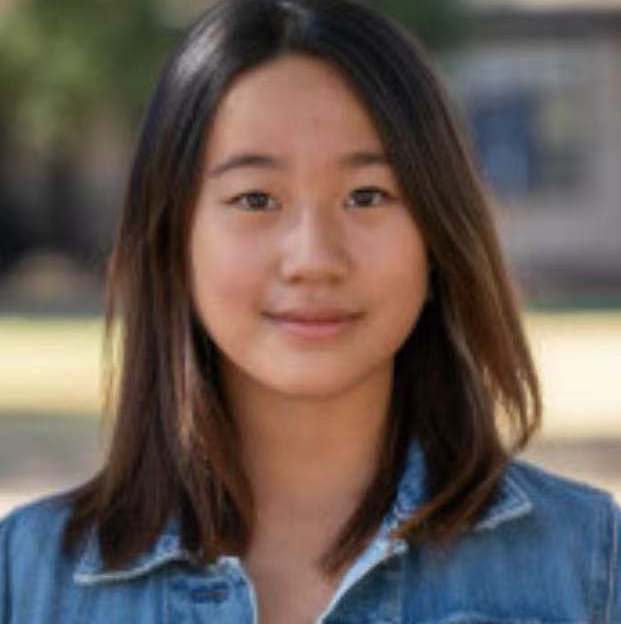
Author: Cathleen Chow
Mentor: Dr. Fabiola Munarin
Cate School
Abstract
While the bone can naturally regenerate itself, in larger bone defects, oftentimes a biomaterial must serve as a scaffold to hold the structure together while the bone regenerates. Calcium phosphates, calcium sulfates, and bioactive glass are common examples of biomaterials that can be used to repair or regenerate the lost or damaged bone. Depending on the situation, the biomaterial may need to be modified to better suit its purpose. This modification can occur solely on the surface (surface modifications) or change the entire material itself (bulk modifications). This paper introduces a few different approaches that have been investigated to improve the biomaterials properties for making them more amenable for bone tissue regeneration.
Introduction
More than 20 million patients worldwide are affected by the loss of bone tissue caused by trauma or disease annually (Iaquinta et al, 2019). The human body can naturally regenerate bone tissue (Dimitriou et al, 2011); however, when such regeneration is needed at a larger scale, surgeons can use a multitude of strategies to address the issue: growth factors such as bone morphogenetic proteins (BMPs), mesenchymal stem cells (MSCs), gene therapy, and biomaterials (Dimitriou et al, 2011). Biomaterials as scaffolds provide a substrate for cell proliferation and adhesion while regulating the cell activity and function–all important factors to induce bone regeneration (Gao et al, 2017). Cell proliferation is the division of cells, a mechanism to induce rapid cell and thus tissue growth. Biomaterials induce cell proliferation through promoting growth factors, proteins that stimulate cell division (Gao et al, 2017). Cell adhesion is organically between cells and cells or cells and the extracellular matrix (ECM), allowing communication and mechanical force to be transferred between the two bodies without separation; in the context of biomaterials, this is important for communication and the clinical performance of the bone, and a cell’s adhesion abilities are usually referred as its biocompatibility (Gao et al, 2017).
Currently, there is no possible way to synthetically produce an entire bone, so biomaterials must be utilized to modify, augment or treat current bone structures (Dimitriou et al, 2011). The biomaterials most commonly used for promoting bone repair include calcium-phosphate ceramics, biopolymers, bioactive glass, calcium phosphates (such as hydroxyapatite),calcium sulphates (such as -tricalcium phosphate), and composite biomaterials (Trimeche, 2017). In developing new biomaterials and strategies to effectively promote bone regeneration, scientists have to meet specific requirements. Function-wise, biomaterials must be osteoinductive (recruitment and proliferation/differentiation of progenitor cells), osteoconductive (bone growth on the biomaterials), and able to perform osseointegration (integration into surrounding bone) (Stevens, 2008). In addition, biomaterials must meet biocompatibility, bioactivity, and controllable biodegradability standards (Gao et al, 2017).
The porous structure of the biomaterial can affect the type, structure, and function of the regenerated tissue (Gao et al, 2017). As the pore allows for cell movement and oxygen flow, depending on the porosity, cells will be restricted or allowed to penetrate, proliferate, and differentiate. Additionally, the rate at which the degradation occurs will vary depending on the size and dispersion of porosity For example, more porous materials favor greater cell growth and transport of nutrients and waste while less porous layers favor external mechanical loading.
There are a few approaches to address the issue of creating optimal biomaterials, including surface and bulk chemical modifications. Surface modifications adjust the physicochemical behavior, interaction attributes, and structural properties of the biomaterial by increasing its compatibility at its surface level (Raval et al, 2019). Conversely, bulk modifications adjust the compatibility of the biomaterial by modifying the entire biomaterial itself, which affects its innate attributes and functions (Raval et al, 2019). Through new developments in technology and the neverending search to modify current biomaterials, scientists aim at developing more types of and more unique uses of biomaterials in the field of bone regeneration.
1. Surface Modifications
The surface of biomaterials are uniquely reactive, often influencing the environment around the biomaterial (Ratner et al, 1996). There are a multitude of ways to measure the distinct characteristics of biomaterial surfaces, of which include contact angle methods, electron spectroscopy, secondary ion mass spectrometry, scanning electron microscopy, and others (Ratner et al, 1996). Surface modifications refers to changes to the outer layer of the biomaterial, involving controlling the surface to modulate its influence and optimize the biomaterial’s compatibility and use (Raval et al, 2019). Surface modifications can be categorized by physical, also called mechanical, and chemical changes (Raval et al, 2019). This section will describe how surface modifications impact on different properties of biomaterials to improve adhesion and biocompatibility to the physiological environment.
1.1 Hydrophilicity and Hydrophobicity
Hydrophilicity refers to when biomaterial surfaces attract water because the surface is polar while hydrophobicity refers to when biomaterial surfaces repel water because the surface is non-polar, and it is measured with the contact angle (hydrophillicity is considered below 90°) (Paterlini et al, 2017). Hydrophilic materials have a much higher wettability, which makes hydrophilic materials a favorable choice through its interaction with the body and improved cell viability and proliferation (Paterlini et al, 2017).
The components of the biomaterial and its hydrophilicity are ultimately dependent on its environment. In a more hydrophobic environment, such as environments with more air, more hydrophobic components may migrate to the surface of the cell; in more hydrophilic environments, such as an aqueous solution, more hydrophilic components would surface (Ratner et al, 1996).
The hydrophilicity and hydrophobicity of a material affect cell behavior. Hydrophobicity increases the stiffness of the biomaterial, and hydrophobic materials are less adhesive and hinders the growth of cells, while hydrophilicity does the opposite (Ferrari et al, 2019). Also, hydrophobic surfaces favor protein absorption while hydrophilic surfaces doesn’t (Paterlini et al, 2017). However, hydrophobic surfaces may induce irreversible absorption of those proteins and even possible denaturation.
Hydrogels are hydrophilic when placed in water, their cross-linked structures begin to swell (Ratner et al, 1996). Its ability to swell, and thus effectively support protein absorption and cell adhesion, makes hydrogel a very common scaffold in bone tissue regeneration (Yue et al, 2020). On the other hand, polytetrafluoroethylene (PTFE) is hydrophobic and thus very lubricant (Ratner et al, 1996). Unlike hydrogels, PTFE does not rely on water to serve its purpose; on the contrary, its repulsion to water actually makes the material more effective.
By changing the surface in such a way to significantly change the contact angle of polymer biomaterials such as polyethylene (PE) or Teflon (FEP), the surfaces of these materials become “super hydrophobic” (Paterlini et al, 2017). Oftentimes, plasma cleaning is used to change the surface of the biomaterial, decreasing in water contact angle and increasing in wettability, thereby making the material more hydrophilic (Ratner et al, 1996). This is specifically done through providing the surface with more “adhesion power and cell proliferation”, making the surface more hydrophilic (Paterlini et al, 2017).
Biodegradable synthetic polymers such as polylactide (PLA) and poly(lactide-co-glycotide) (PLGA) are common orthopedic biomaterials, but are not bioactive enough nor induce enough cell regeneration. Thus, researchers have modified the surfaces of many biodegradable synthetic polymers to improve their biological response, including active gases (radiation or vapor), plasma treatment, layer-by-layer deposition, or self-assembled monolayers (Paterlini et al, 2017). By making the surface more porous or more hydrophobic, biodegradable synthetic polymers, when used, have increased cell adhesion and proliferation (Paterlini et al, 2017).
Depending on the situation, making a surface more hydrophobic or more hydrophilic can help serve its purpose better. However, either feature has its advantages and disadvantages, and many other modifications are used to combat the effects of a biomaterial’s hydrophilicity/hydrophobicity.
1.2 Peptide Binding
An alternative to artificial biomaterials such as ceramics and synthetic polymers, natural biomaterials, namely natural polymers, are known to be more biocompatible, more biodegradable, and have no toxicity whilst still efficiently engineering a bone graft (Guo et al, 2021). They are most commonly categorized as either protein-based biomaterials (such as collagen or gelatin) or polysaccharide-based biomaterials (such as hyaluronan or cellulose) (Chen et al, 2015). However, peptide-based biomaterials are less frequently used for bone regeneration (Collier et al, 2011).
Between protein-based and peptide-based biomaterial, peptide-based biomaterials can in fact be a better alternative for it is more chemically defined, meaning that it is easier to modify precisely (Collier et al, 2011). When using protein-based biomaterials, contaminants like lipopolysaccharide often affect the effectiveness of the biomaterial. The chemical definition and precision of peptides makes it easier to take away those contaminants (Collier et al, 2011). Furthermore, using peptide sequences in enzyme substrate sequences help precisely control the degradation of the matrices itself, suggesting that modifying a biomaterial using peptides is an efficient way to control the biodegradability of the material (Collier et al, 2011). Peptides may be conjugated to a biomaterial through specific chemistries and use of varying crosslinkers (Collier et al, 2011).
Furthermore, peptides are highly capable of binding to growth factors, which are proteins vital to the regeneration of bone (Collier et al, 2021).
The most studied peptides that are used with biomaterials for biomedical applications are RGD, dominant in fibronectin, and YIGSR, dominant in laminin, two of the main components of the extracellular matrix (ECM).Though many studies have demonstrated improved regeneration and cell adhesion when coupling growth factors with biomaterials (Collier et al, 2011), it is important to note that the RGD peptide in modification to a common natural polymer hydroxyapatite (which is actually found in bone itself) actually resulted in little benefits and was worse off for the regeneration process by inhibiting bone formation (Hennessy et al, 2009).
However, RGD as a modification to polycaprolactone (PCL) two-dimensional film has improved bone marrow stromal cell (BMSC) adhesion to the films because of its induced cell adhesion and thus cell-substrate interaction. PCL is a biomaterial very suited for the needs of tissue engineering and bone regeneration primarily because of its mechanical properties, affordability, and fabrication with proper porosity (Zhang et al, 2009). PCL lacks proper cell adhesion due to its hydrophobicity, which is a modification that RGD helps enhance. For three-dimensional PCL scaffolds, the RGD was crosslinked to modify the scaffold and, despite resulting in inconsistent cell distribution, still improved BMSC adhesion (Zhang et al, 2009).
Thus, in consideration of RGD as a modification in theory and in practice for two different biomaterials, RGD, while flawed, is a modification with much potential and could perhaps even be re-introduced for future biomaterials.
1.3 Calcium Phosphate Coatings
A common strategy used primarily in orthopedics and dentistry, calcium phosphate coatings are a surface modification evolved from the traditional calcium phosphate ceramic materials (CPC). Calcium phosphates are primarily coated onto metal biomaterials, such as titanium and magnesium (Jeong et al, 2019). Calcium phosphate appeals as an orthopedic biomaterial modification primarily because of its quick and strong bond and integration to bone tissue (Yoshinari et al, 2002). Furthermore, the coatings are often applied for increased bioactivity and bone regeneration (Jeong et al, 2019). Calcium phosphate is very porous, which helps contact with physiologic fluids and protein absorption. Moreover, depending on the pore size, calcium phosphates have been shown to induce bone ingrowth and angiogenesis (Jeong et al, 2019).
Sol-gel and electrodeposition methods are often used to coat surfaces with calcium phosphates (Jeong et al, 2019). Physical vapor deposition (PVD), ion sputtering, and ion plating are also techniques to adhere a thin even coat of the calcium phosphate. The ion beam dynamic mixing (IBDM) method combines PVD and ion implantation not only provides a thin even coat of the calcium phosphate, but also has a higher deposition rate and better adhesion qualities (Yoshinari et al, 2002). Calcium phosphates have tendencies to be amorphous (Yoshinari et al, 2002). Referred to as ACP (amorphous calcium phosphate), ACP is a hydrated, loosely structured, and often unstable biomaterial (Habraken et al, 2016). However, because of the effectiveness of ACP as a biomaterial for biological pathways and bone regrowth, namely being a potential precursor for bone growth as having similar chemical composition of bone mineral, a solution has been found (Habraken et al, 2016). Specifically, the amorphousness of ACP is mitigated by rapid infrared heat-treatment using hydroxyapatite. (Yoshinari et al, 2002).
2. Bulk Modifications
Bulk modifications most commonly refer to the chemical modifications to a material, affecting both the material core in addition to the surface. Unlike surface modifications, where the improvement is uniquely achieved on a small superficial layer, bulk modifications often change the properties of the material through tweaking its chemical composition. This section focuses on different properties that can be achieved with bulk chemical modifications.
2.1 Degradability
The degradability of a biomaterial, or biodegradability, refers to a material’s ability to break down chemically into non-toxic components. And, depending on the application of the material, the implanted biomaterial could stay stable in the system for long periods of time or be rapidly dissolved in its environment. A too fast degrading biomaterial may result in a collapsed porous structure, no mass transfer, and thus necrosis (cell death); on the contrary, a too slow degrading biomaterial may cause a fibrotic encapsulation, which would hinder tissue regeneration (Park et al, 2010). Degradable biomaterials can help scaffold the defected bone area while new bone tissue forms and eventually seamlessly replaces the biomaterial as the biomaterial degrades without producing toxic (Davison et al, 2014).
A well-known biodegradable and biocompatible biomaterial is chitosan, a natural polymer that, because of its porosity, helps in the bone implantation process (Zhang et al, 2019). Its biodegradability is dependent on its degree of deacetylation, or the amount of glucosamine in the chitosan; highly deacetylated chitosan degrades much slower than less deacetylated chitosan (Zhang et al, 2019). In this sense, the content of glucosamine can be manipulated to control the biodegradability of the chitosan (Zhang et al, 2019).
Alginate is a natural polymer that is biodegradable, non-toxic, can become gel, biocompatible, and easy to process (Sahoo et al, 2021). However, despite being widely researched and used for its use as a drug delivery and being cell carriers, it rapidly degrades (Birdi et al, 2011). This makes alginate harder to use as an orthopedic biomaterial since bone regeneration often takes a long time to occur. Through the addition of orthosilicic acid (OSA), an alginate hydrogel was able to slow down degradation in tests using a potent calcium chelator (EDTA) (Birdi et al, 2011). Through these tests, the OSA integrated alginate hydrogel resulted in no detect of calcium release than alginate hydrogel alone (which resulted in significant calcium release), suggesting that OSA inhibits the release of calcium through forming calcium cross-links, thus resulting in a slower degradation speed (Birdi et al, 2011).
When developing new composite biomaterials, biomaterials, particularly polymers, are chosen based on biodegradability, biocompatibility, and mechanical strength. For this reason, the polymers are commonly PLA or PGA polymers. PCL is also becoming more common for long-term bone regeneration due to its low degradation rate, being biocompatible, and mechanical strength. Together with zein through solvent casing particle leaching (SCPL), the zein/PCL composite scaffold’s degradability is controlled by zein (Zhu et al, 2020).
2.2 Drug Release
While most biomaterials in bone regeneration serve as a scaffold for the bone to regenerate by itself, some biomaterials have been modified to serve as a drug carrier to quicken the regeneration process. However, drug delivery has been historically hard to implement; drug are commonly delivered through a noninvasive systematic delivery, but this may result in a poor distribution of the drug throughout the bone (Newman et al, 2016). By using carriers such as peptides, aptamers, and phosphate-rich compounds—all materials that are biocompatible with the environment—specific to proteins and other cells unique to the environment, these carriers can deliver the drugs with great distribution while maintaining its systematic function (Newman et al, 2016). Also, these carriers further support on-demand drug delivery and tissue engineering (Vashist et al, 2017).
An example of an excellent biopolymer with drug release is elastin-like polypeptides (ELP), which is based off of human elastic (MacEwan et al, 2014). Specifically for bones, coacervate ELP nanoparticles physically encapsulate BMP-2 and BMP-14 cytokines, growth factors that induce bone growth (MacEwan et al, 2014).
Using biomaterials as drug carriers requires the biomaterial chemical composition to be adjusted to hold onto the drug. Hydrogels are commonly used for scaffold due to their properties being similar to that of the extracellular matrix of organs or tissue. It fulfills the role as a scaffold because of its mechanical strength, porosity, and diffusive properties. In the field of nanomedicine, hydrogels are now being developed into injectable hydrogels, in which hydrogels serve as a drug carrier that can be made into a desired shape depending on the function of the biomaterial. This was made through a series of polymers and live cells, which formed a hydrogel depot which was processed using the sol-gel method. By making the hydrogel injection, it not only served as a drug carrier, but also a sealing agent, tissue-engineering product, and surgical glue (Vashist et al, 2017).
Electrospun nanofibers are seen as great drug carriers due to their high surface area and mechanical properties, depending on what polymer the nanofibers are derived from. Silk fibroin protein has also become a common drug carrier partly due to its purification, sterilization, lack of cross-linkers, and great biocompatibility amongst other qualifications (Farokhi et al, 2020).
Amphiphilic block copolymers (ABC) is commonly applied in the pharmaceutical field and extensively used as a drug carrier (Adams et al, 2005), and is also being researched as a modification to scaffolds to induce their drug delivery abilities (Kutikov, 2015). Polyesters, while great as scaffold, contain many limitations due to their hydrophobicity, with drug delivery being one of them (Kutikov, 2015). By integrating hydrophobic poly(ethylene glycol) (PEG) blocks with hydrophobic polyesters to become an ABC, the characters of the copolymer completed shifted from its physical properties to biological performance, with positive but not conclusive results in drug delivery for the repair of bone tissue (Kutikov, 2015).
While drug delivery as a bulk modification is still being researched, drug delivery systems in biomaterials that naturally contain them are essential to inducing bone growth.
Conclusion
In this review paper, different properties of both surface and bulk modifications in biomaterials primarily used for bone regeneration were assessed. While these are the most common properties, there are far more to be looked at and each property is far more complex than the overview and insight that this paper provides. However, this paper does provide a holistic view on the innovative aspect of new techniques and primary goals in biomaterials for bone regeneration.
References
Adams, M. L., Lavasanifar, A., & Kwon, G. S. (2003). Amphiphilic block copolymers for drug delivery. Journal of Pharmaceutical Sciences, 92(7), 1343-1355. doi:10.1002/jps.10397
Birdi, G., Bridson, R. H., Smith, A. M., Mohd Bohari, S. P., & Grover, L. M. (2012). Modification of alginate degradation properties using orthosilicic acid. Journal of the Mechanical Behavior of Biomedical Materials, 6, 181-187. doi:https://doi.org/10.1016/j.jmbbm.2011.10.001
Bose, S., Robertson, S. F., & Bandyopadhyay, A. (2018). Surface modification of biomaterials and biomedical devices using additive manufacturing. Acta Biomaterialia, 66, 6-22. doi:10.1016/j.actbio.2017.11.003
Chen, F., & Liu, X. (2016). Advancing biomaterials of human origin for tissue engineering. Progress in Polymer Science, 53, 86-168. doi:10.1016/j.progpolymsci.2015.02.004
Collier, J. H., & Segura, T. (2011). Evolving the use of peptides as biomaterials components
Davison, N. L., Barrère-de Groot, F., Grijpma, D. W., van Blitterswijk, C. A., & de Boer, J. (2015). Degradation of biomaterials. Tissue engineering (pp. 177-215) Academic Press. Retrieved from https://www.narcis.nl/publication/RecordID/oai:ris.utwente.nl:publications%2Fb23e056f-d247-41bd-9a68-71fa6afe113a
Dimitriou, R., Jones, E., McGonagle, D., & Giannoudis, P. V. (2011). Bone regeneration: Current concepts and future directions. BMC Medicine, 9(1), 66. doi:10.1186/1741-7015-9-66
Eliaz, N., & Metoki, N. (2017). Calcium phosphate bioceramics: A review of their history, structure, properties, coating technologies and biomedical applications. Materials, 10(4), 334. doi:10.3390/ma10040334
Elwing, H. (1998). Protein absorption and ellipsometry in biomaterial research. Biomaterials, 19(4), 397-406. doi:10.1016/S0142-9612(97)00112-9
Farokhi, M., Mottaghitalab, F., Reis, R. L., Ramakrishna, S., & Kundu, S. C. (2020). Functionalized silk fibroin nanofibers as drug carriers: Advantages and challenges. Journal of Controlled Release, 321, 324-347. doi:https://doi.org/10.1016/j.jconrel.2020.02.022
Ferrari, M., Cirisano, F., & Morán, M. C. (2019). Mammalian cell behavior on hydrophobic substrates: Influence of surface properties doi:10.3390/colloids3020048
Gao, C., Peng, S., Feng, P., & Shuai, C. (2017a). Bone biomaterials and interactions with stem cells. Bone Research, 5(4), 253-285. doi:10.1038/boneres.2017.59
Gao, C., Peng, S., Feng, P., & Shuai, C. (2017b). Bone biomaterials and interactions with stem cells. Bone Research, 5(1), 17059. doi:10.1038/boneres.2017.59
Girón, J., Kerstner, E., Medeiros, T., Oliveira, L., Machado, G. M., Malfatti, C. F., et al. (2021). Biomaterials for bone regeneration: An overview. Brazilian Journal of Medical and Biological Research, 54(9), e11055. doi:10.15761
Gough, J. (2011). 12 – modifying biomaterial surfaces to optimise interactions with soft tissues. In R. Williams (Ed.), Surface modification of biomaterials (pp. 309-325) Woodhead Publishing. doi:https://doi.org/10.1533/9780857090768.2.309
Guo, L., Liang, Z., Yang, L., Du, W., Yu, T., Tang, H., et al. (2021). The role of natural polymers in bone tissue engineering. Journal of Controlled Release : Official Journal of the Controlled Release Society, 338, 571-582. doi:S0168-3659(21)00469-7 [pii]
Habraken, W., Habibovic, P., Epple, M., & Bohner, M. (2016). Calcium phosphates in biomedical applications: Materials for the future? Materials Today (Kidlington, England), 19(2), 69-87. doi:10.1016/j.mattod.2015.10.008
Hennessy, K. M., Clem, W. C., Phipps, M. C., Sawyer, A. A., Shaikh, F. M., & Bellis, S. L. (2008). The effect of RGD peptides on osseointegration of hydroxyapatite biomaterials. Biomaterials, 29(21), 3075-3083. doi:10.1016/j.biomaterials.2008.04.014
Iaquinta, M. R., Mazzoni, E., Manfrini, M., D’Agostino, A., Trevisiol, L., Nocini, R., et al. (2019). Innovative biomaterials for bone regrowth. International Journal of Molecular Sciences, 20(3), 618. doi:10.3390/ijms20030618
Jeong, J., Kim, J. H., Shim, J. H., Hwang, N. S., & Heo, C. Y. (2019). Bioactive calcium phosphate materials and applications in bone regeneration. Biomaterials Research, 23(1), 4. doi:10.1186/s40824-018-0149-3
Juhasz, J. A., & Best, S. M. (2011). 6 – surface modification of biomaterials by calcium phosphate deposition. In R. Williams (Ed.), Surface modification of biomaterials (pp. 143-169) Woodhead Publishing. doi:https://doi.org/10.1533/9780857090768.1.143
Kearns, V. R., Mcmurray, R. J., & Dalby, M. J. (2011). 7 – biomaterial surface topography to control cellular response: Technologies, cell behaviour and biomedical applications. In R. Williams (Ed.), Surface modification of biomaterials (pp. 169-201) Woodhead Publishing. doi:https://doi.org/10.1533/9780857090768.1.169
Kim, H. J., You, S. J., Yang, D. H., Eun, J., Park, H. K., Kim, M. S., et al. (2020). Injectable hydrogels based on MPEG-PCL-RGD and BMSCs for bone tissue engineering. Biomaterials Science, 8(15), 4334-4345. doi:10.1039/d0bm00588f
Kumar, N., Ravikumar, M. N. V., & Domb, A. J. (2001). Biodegradable block copolymers. Advanced Drug Delivery Reviews, 53(1), 23-44. doi:https://doi.org/10.1016/S0169-409X(01)00219-8
Kutikov, A. B., & Song, J. (2015). Biodegradable PEG-based amphiphilic block copolymers for tissue engineering applications. ACS Biomaterials Science & Engineering, 1(7), 463-480. doi:10.1021/acsbiomaterials.5b00122
Liu, J., Yao, Y., Li, X., & Zhang, Z. (2021). Fabrication of advanced polydimethylsiloxane-based functional materials: Bulk modifications and surface functionalizations. Chemical Engineering Journal, 408, 127262. doi:https://doi.org/10.1016/j.cej.2020.127262
Lu, J., Yu, H., & Chen, C. (2018). Biological properties of calcium phosphate biomaterials for bone repair: A review. RSC Advances, 8(4), 215-233. doi:10.1039/c7ra11278e
MacEwan, S. R., & Chilkoti, A. (2014). Applications of elastin-like polypeptides in drug delivery. Journal of Controlled Release, 190, 314-330. doi:10.1016/j.jconrel.2014.06.028
Matassi, F., Nistri, L., Chicon Paez, D., & Innocenti, M. (2011). New biomaterials for bone regeneration. Clinical Cases in Mineral and Bone Metabolism : The Official Journal of the Italian Society of Osteoporosis, Mineral Metabolism, and Skeletal Diseases, 8(1), 21-24. Retrieved from PubMed database. Retrieved from https://pubmed.ncbi.nlm.nih.gov/22461799 https://www.ncbi.nlm.nih.gov/pmc/articles/PMC3230919/
Mateos-Timoneda, M. A., Planell, J. A., & Engel, E. (2011). 13 – modifying biomaterial surfaces for the repair and regeneration of nerve cells. In R. Williams (Ed.), Surface modification of biomaterials (pp. 325-343) Woodhead Publishing. doi:https://doi.org/10.1533/9780857090768.2.325
Meyers, S. R., Khoo, X., Huang, X., Walsh, E. B., Grinstaff, M. W., & Kenan, D. J. (2008). The development of peptide-based interfacial biomaterials for generating biological functionality on the surface of bioinert materials. Biomaterials, 30(3), 277-286. doi:10.1016/j.biomaterials.2008.08.042
Murthy, N. S. (2011). 9 – techniques for analyzing biomaterial surface structure, morphology and topography. In R. Williams (Ed.), Surface modification of biomaterials (pp. 232-255) Woodhead Publishing. doi:https://doi.org/10.1533/9780857090768.2.232
Newman, M. R., & Benoit, D. S. (2016). Local and targeted drug delivery for bone regeneration. Current Opinion in Biotechnology, 40, 125-132. doi:10.1016/j.copbio.2016.02.029
Park, S., Gil, E. S., Shi, H., Kim, H. J., Lee, K., & Kaplan, D. L. (2010). Relationships between degradability of silk scaffolds and osteogenesis. Biomaterials, 31(24), 6162-6172. doi:10.1016/j.biomaterials.2010.04.028
Paterlini, T. T., Nogueira, L. F. B., Tovani, C. B., Cruz, M. A. E., Derradi, R., & Ramos, A. P. (2017). The role played by modified bioinspired surfaces in interfacial properties of biomaterials. Biophysical Reviews, 9(5), 683-698. doi:10.1007/s12551-017-0306-2
Ratner, B. D., Hoffman, A. S., Schoen, F. J., & Lemons, J. E. (2012). Biomaterials science. San Diego: Elsevier Science & Technology. Retrieved from https://ebookcentral.proquest.com/lib/[SITE_ID]/detail.action?docID=1074425
Raval, N., Kalyane, D., Maheshwari, R., & Tekade, R. K. (2019). Chapter 17 – surface modifications of biomaterials and their implication on biocompatibility. In R. K. Tekade (Ed.), Biomaterials and bionanotechnology (pp. 639-674) Academic Press. doi:https://doi.org/10.1016/B978-0-12-814427-5.00017-2
Rhodes, A., Sandhu, S. S., & Onis, S. J. (2011). 2 – surface modification of biomaterials by covalent binding of poly(ethylene glycol) (PEG). In R. Williams (Ed.), Surface modification of biomaterials (pp. 39-55) Woodhead Publishing. doi:https://doi.org/10.1533/9780857090768.1.39
Sahoo, D. R., & Biswal, T. (2021). Alginate and its application to tissue engineering. SN Applied Sciences, 3(1) doi:10.1007/s42452-020-04096-w
Sammons, R. L. (2011). 15 – modifying biomaterial surfaces to optimise interactions with bone. In R. Williams (Ed.), Surface modification of biomaterials (pp. 365-400) Woodhead Publishing. doi:https://doi.org/10.1533/9780857090768.2.365
Smith, K. H., & Haycock, J. W. (2011). 14 – modifying biomaterial surfaces to control stem cell growth and differentiation. In R. Williams (Ed.), Surface modification of biomaterials (pp. 344-364) Woodhead Publishing. doi:https://doi.org/10.1533/9780857090768.2.344
Spicer, C. D., Pashuck, E. T., & Stevens, M. M. (2018). Achieving controlled Biomolecule–Biomaterial conjugation. Chemical Reviews, 118(16), 7702-7743. doi:10.1021/acs.chemrev.8b00253
Stevens, M. M. (2008). Biomaterials for bone tissue engineering. Materials Today, 11(5), 18-25. doi:https://doi.org/10.1016/S1369-7021(08)70086-5
Szili, E. J., Short, R. D., Steele, D. A., & Bradley, J. W. (2011). 1 – surface modification of biomaterials by plasma polymerization. In R. Williams (Ed.), Surface modification of biomaterials (pp. 3-39) Woodhead Publishing. doi:https://doi.org/10.1533/9780857090768.1.3
Wei, S., Ma, J., Xu, L., Gu, X., & Ma, X. (2020). Biodegradable materials for bone defect repair. Military Medical Research, 7(1), 54. doi:10.1186/s40779-020-00280-6
Yoshinari, M., Oda, Y., Inoue, T., Matsuzaka, K., & Shimono, M. (2002a). Bone response to calcium phosphate-coated and bisphosphonate-immobilized titanium implants. Biomaterials, 23(14), 2879-2885. doi:https://doi.org/10.1016/S0142-9612(01)00415-X
Yoshinari, M., Oda, Y., Inoue, T., Matsuzaka, K., & Shimono, M. (2002b). Bone response to calcium phosphate-coated and bisphosphonate-immobilized titanium implants. Biomaterials, 23(14), 2879-2885. doi:https://doi.org/10.1016/S0142-9612(01)00415-X
Yue, S., He, H., Li, B., & Hou, T. (2020). Hydrogel as a biomaterial for bone tissue engineering: A review. Nanomaterials (Basel, Switzerland), 10(8), 1511. doi:10.3390/nano10081511
Zhang, H., Yang, L., Yang, X., Wang, F., Feng, J., Hua, K., et al. (2019). Demineralized bone matrix carriers and their clinical applications: An overview. Orthopaedic Surgery, 11(5), 725-737. doi:10.1111/os.12509
Zhang, H., Lin, C., & Hollister, S. J. (2009). The interaction between bone marrow stromal cells and RGD-modified three-dimensional porous polycaprolactone scaffolds. Biomaterials, 30(25), 4063-4069. doi:10.1016/j.biomaterials.2009.04.015
About the author
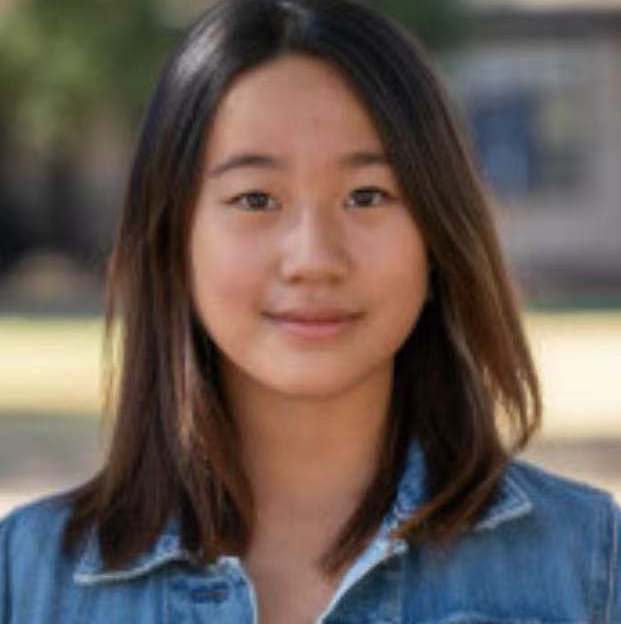
Cathleen Chow
Cathleen is currently a junior at the Cate School in Carpinteria, California. She loves playing volleyball and waterpolo and is on the school varsity team. In addition, Cathleen plays the violin and you’ll frequently find her in the studio practicing for an upcoming chamber piece. Despite her numerous interests, she always makes time to research and learn more about the sciences that she finds fascinating and the sciences that affect our daily lives.