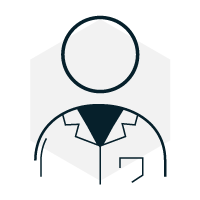
Author: Harsheel Dhruva
Mentor: Dr. Fabiola Munarin
Irvington High School
Abstract
The ability of stem cells to self-renew and form different mature cells expands the possibilities of application in cell therapy, such as tissue reorganization in regenerative medicine, drug detection, and treatment of neurodegenerative diseases. In addition to stem cells found in embryos, several adult organs and tissues also have stem cell niches in an undifferentiated state. In the central nervous system of adult mammals, neurogenesis occurs in two areas: the subventricular zone and the dentate gyrus of the hippocampus. The different nervous systems originate from adult neural stem cells, which can self-renew or differentiate into astrocytes, oligodendrocytes, or neurons that respond to specific stimuli. The regulation of the fate of neural stem cells is a finely controlled process that relies on a complex regulatory network that extends from the epigenetics to the translational level and involves components of the extracellular matrix. Therefore, a better understanding of the mechanisms by which neurogenesis is induced, regulated, and maintained will provide clues for the development of new strategies for neurodegenerative treatment. In this review, we focus on the regulatory mechanisms of transcription factors, microRNAs, and components of the extracellular matrix in neuronal differentiation.
1. Introduction
Neocortical circuits consist of profoundly interconnected excitatory glutamatergic and inhibitory GABAergic neurons, which are produced from unmistakable pools of RGCs. During embryo development, the excitatory neurons are formed from RGCs confined in the ventricular zone of the dorsal telencephalon and relocate radially toward the pial surface in a back to the front way (spiral movement). Then again, inhibitory neurons fundamentally begin from the ventral telencephalon and relocate digressively into the neocortex (distracting movement). Regardless of such unique formative starting points, both excitatory and inhibitory neurons go through the multipolar stage with a few minor cycles in the neocortex before axon augmentation. Then, at that point, they go through dynamic morphological changes to start axon development, in particular, neuronal polarization. Sakakibara and Hatanaka () assessed the consecutive occasions in polarization cycles of both excitatory and inhibitory neurons, and they talked about the hidden atomic instruments. At the multipolar stage, the excitatory neurons fleetingly utilize a multipolar relocation mode, to be specific movement with no fixed heading, in the subventricular and middle of the road zones. Then, at that point, they receive a bipolar shape during neuronal polarization and relocate rapidly toward the pial surface along with RGC measures, which is called headway mode.
Many types of molecules are involved in these powerful changes in neuron morphology and migration. The small GTP binding protein plays an important role in regulating the development of the cortex and the formation of neurons. The Rnd protein, “abnormal” relatives of Rho, was displayed in neuronal movement, and their ascending and descending pathways were discussed. Many cytoplasmic protein elements, including parts of the cytoskeleton, are managed through phosphorylation and dephosphorylation measures. Ohshima focused on protein kinases, including CDK5 and JNK, and examined their paperwork on cytoskeletal associations during multipolar bipolar progression and extended movement. OhtakaMaruyama and Okado comprehensively summarized the atomic pathways involved in these formation cycles and emphasized the importance of subplate neurons in steering events and the development of the six-layer neocortex design.
Pluripotent stem cells are usually derived from embryonic tissue. At least three different types of mammalian pluripotent stem cells have been identified: embryos or cancer cells (CE), embryonic stem cells (ES) derived from the inner cell mass of blastocysts, and embryonic germ cells (EG) derived from post-implantation embryos. In the early 1990s, several groups reported the existence of a subset of stem cells found in the central nervous system (CNS). These cells form the brains of fetal mice or mice that grow in culture and show an almost unlimited lifespan. However, compared to embryonic stem cells, their differentiation potential is more limited and they mainly produce three main cell types of the central nervous system: neurons, astrocytes, and oligodendrocytes, hence the name NSC. These cells have also been isolated from the adult central nervous system, although it is not clear whether these dividing cells are truly pluripotent or whether their fate becomes more restricted during development. For the purposes of this review, NSCs are defined as nerve cells that have the potential to self-renew and generate all the different types of cells in the nervous system after differentiation.
2. Method
Neuronal differentiation is an early event in mammalian embryogenesis, occurring shortly after germ layer differentiation. The organization of the central nervous system is derived from a well-defined neuroectoderm, the neural plate, which is located in the dorsal midline of the embryo. It appears that neural plaque is produced by signals that locally inhibit or avoid inducing non-neural differentiation. Examples of such signals come from bone morphogenetic protein (BMP) and other molecules of the transforming growth factor-beta (TGF beta) superfamily, which direct epidermal differentiation when gastrulation
Neuronal fate is inhibited by BMP. In the body, several molecules that promote neuronal differentiation, such as noggin, follistatin, and chordates, are antagonists of BMP. Although noggin antagonizes BMP signaling, it is not necessary for the induction of early neurogenesis, because knockout mice are normal at embryonic day 8.5 (although they die at birth). These data indicate that other BMP antagonists can compensate without Noggin expression and illustrate the concept of redundant signaling pathways during embryonic development. The role of BMP is further regulated by the presence of two ubiquitously expressed BMP receptors, BMPR1A and BMPR1B, which do not appear until the 9th day of the embryo. The expression of BMPR1A induces the expression of BMPR1B, and this process is inhibited by the sonic hedgehog. The further development of the neural lamina into mature cells of the central nervous system is clearly and precisely regulated by spatial and temporal differentiation patterns. The growth and proliferation of cells in the early neural plate eventually lead to the closure of the developing nerve sulcus and the formation of a hollow neural tube. The neural tube cavity produces the ventricular system and the epithelial layer contains stem cells that will produce neurons and glial cells of the central nervous system. One of the central problems in developmental neurobiology is the mechanism by which a simple neuroepithelium (only one cell thick) can produce the various cell types that make up the mammalian central nervous system. Currently, there is a large number of studies that have determined the internal factors and external soluble signals that affect this regional pattern and specific neural differentiation. An example of this invertebrate neurogenesis is the transmission of dorsal and ventral patterns of opposing soluble signals: sonic hedgehog (Shh) and BMP antagonists, chordin and noggin, are secreted from the bottom plate, while other signals are emitted from the top plate. Form a gradient. Signal concentration. The precise concentration and ratio of each signal in the neural tube is critical to the development of specific neuronal phenotypes at different points along the gradient. For example, there is a concentration-dependent induction of model genes in progenitor cells encoding homeodomain transcription factors. These differences in expression patterns produce neuronal clusters with different division and differentiation patterns (and ultimately different phenotypes) along the dorsal-ventral axis of the plaque. In humans, the neural tube is formed during the third and fourth weeks of pregnancy. Initially, the neuroepithelial lining consisted of a single layer of neural stem cells with similar morphology. These cells then divide symmetrically to enrich the pool of NSCs or divide asymmetrically to produce a more differentiated progeny from which neurons and mature cells of the glial mass line develop. Although the BMP family of molecules may be involved again, the signals that determine symmetric or asymmetric division are not yet fully understood.
Retroviral marker studies have been used to identify dividing cells in the ventricular region. Approximately 48% of the labeled cells remained in the colonies in the ventricular zone, indicating self-renewal at this site. In humans and other mammals, the active proliferation of such progenitor cells is likely to be balanced by apoptosis to maintain a stable population, but the exact mechanism has not been determined. As development continues, neurons migrate, partly guided by radially oriented glial processes, and the size of the ventricular area decreases. Neural stem cells are still attached to the basal layer. It has been shown that these cells can divide asymmetrically, and the more differentiated offspring migrate from the ventricular area to the overlying cortex. By obtaining specific phenotypic markers, these cells can divide further and distinguish them from neural stem cells. During CNS development, the temporal pattern causes oligodendrocytes to generate neuronal cell types earlier. In the spinal cord, these two cell populations appear to be produced from a common precursor, and the final decision of fate depends on external signals and specific patterns of transcriptional activation. There are two main types of transcription factors that can determine the fate of neurons or the glia. These are homeodomain factors, an example is NKx2.2, and the basic helix loop helix family of transcription factors, including Olig1 and Olig2. The expression of Olig transcription factors is regulated by external signals (such as Shh), and their expression has been shown to clearly define cells as oligodendrocytes. However, in mice, Olig1 and Olig2 are expressed from E9, long before the presence of oligodendrocyte precursors. At this early stage, Olig2 is known to be the core of neuronal development in specific areas of the ventral spinal cord and is found in cells that can track the fate of motor neurons. The importance of Olig2 in neurodevelopment has been demonstrated in functional gain experiments. As development proceeded, the expression of Olig1 and Olig2 persisted and began to overlap with the homeodomain transcription factor NKx2.2. These doubly positive progenitor cells migrate from the ventral midline and mature into oligodendrocytes. Olig1 and Olig2 have been developed in double-mutant mice. These animals lack oligodendrocytes and also have a considerable loss of motor neurons. In the Olig1 / Olig2 double mutant, the offspring of stem cells in the pMN region of the developing spinal cord generally develop into motor neurons, then oligodendrocytes, but form V2 interneurons and then astrocytes. These results indicate that the expression of the combination of transcription factors determines the fate of the stem cell bank in the developing embryo. However, this does not rule out the existence of more restrictive dividing cell populations. The expression of proglia transcription factors can be regulated by cell surface receptors such as gaps. The jagged1 (notched ligand) signal of neurons has been shown to inhibit the phenotype of oligodendrocytes. Presumably, when the number of neurons is sufficient, jagged1 is down-regulated, and a signal from the original oligodendrocytes (one of which may increase electrical activity) triggers myelination. Once the neural precursor determines the fate of the oligodendrocyte lineage, the last step of honey-forming cell formation needs to exist.
3. Conclusion
All in all, corticogenesis from mouse ESCs shows a setup collection of parent-of-beginning articulation and DNA methylation of engraved loci. This model could be utilized dependably to unwind the atomic components engaged with choosing the communicated parental allele with regard to engraving during the cortical turn of events. Our discoveries likewise give support to utilize ESCs to demonstrate cortical turn of events and for drug screening. The in vitro corticogenesis framework could be an integral asset to pinpoint sedates that de-curb the quieted parental allele in specific mind sicknesses related to irritated IG articulation or to gauge the effect of ecotoxic compounds on the epigenetic marks and the advancement of cortical cells. The cerebral cortex creates through the organized age of many neuronal subtypes, yet the systems included stay hazy. Here we show that mouse undeveloped undifferentiated organisms, refined with no morphogen except for within the sight of a sonic hedgehog inhibitor, summarize in vitro the significant achievements of cortical turn of events, prompting the successive age of a different collection of neurons that show the most notable elements of veritable cortical pyramidal neurons. When joined into the cerebral cortex, these neurons foster examples of axonal projections relating to a wide scope of cortical layers, yet in addition to exceptionally explicit cortical regions, specifically visual and limbic regions, subsequently showing that the character of a cortical region can be determined with no impact from the mind. The disclosure of natural corticogenesis reveals new insight into the instruments of neuronal particular and opens new roads for the demonstrating and treatment of neural disorder.
Works Cited
- MuhChyi C, Juliandi B, Matsuda T, Nakashima K. Epigenetic regulation of neural stem cell fate during corticogenesis. Int J Dev Neurosci. 2013 Oct;31(6):424-33. doi: 10.1016/j.ijdevneu.2013.02.006. Epub 2013 Mar 4. PMID: 23466416.
- Johnson CA, Wright CE, Ghashghaei HT. Regulation of cytokinesis during corticogenesis: focus on the midbody. FEBS Lett. 2017 Dec;591(24):4009-4026. doi: 10.1002/1873-3468.12676. Epub 2017 Aug 21. PMID: 28493553.
- Tabata H. Diverse subtypes of astrocytes and their development during corticogenesis. Front Neurosci. 2015 Apr 7;9:114. doi: 10.3389/fnins.2015.00114. PMID: 25904839; PMCID: PMC4387540.
- Pilaz LJ, Silver DL. Post-transcriptional regulation in corticogenesis: how RNA-binding proteins help build the brain. Wiley Interdiscip Rev RNA. 2015 Sep-Oct;6(5):501-15. doi: 10.1002/wrna.1289. Epub 2015 Jun 18. PMID: 26088328; PMCID: PMC4624281.
- Agirman G, Broix L, Nguyen L. Cerebral cortex development: an outside-in perspective. FEBS Lett. 2017 Dec;591(24):3978-3992. doi: 10.1002/1873-3468.12924. Epub 2017 Dec 14. PMID: 29194577.
- Varrault A, Journot L, Bouschet T. Cerebral Cortex Generated from Pluripotent Stem Cells to Model Corticogenesis and Rebuild Cortical Circuits: In Vitro Veritas? Stem Cells Dev. 2019 Mar 15;28(6):361-369. doi: 10.1089/scd.2018.0233. Epub 2019 Feb 20. PMID: 30661489.
- Zhu X, Wu Y, Li C, Yan W, Pan J, Wang S, Zhao S. Prenatal Exposure to Gossypol Impairs Corticogenesis of Mouse. Front Neurosci. 2020 Apr 3;14:318. doi: 10.3389/fnins.2020.00318. PMID: 32317927; PMCID: PMC7146080.
- Baum T, Gama V. Dynamic properties of mitochondria during human corticogenesis. Development. 2021 Feb 19;148(4):dev194183. doi: 10.1242/dev.194183. PMID: 33608250; PMCID: PMC7903999.
- Lee CT, Bendriem RM, Wu WW, Shen RF. 3D brain Organoids derived from pluripotent stem cells: promising experimental models for brain development and neurodegenerative disorders. J Biomed Sci. 2017 Aug 20;24(1):59. doi: 10.1186/s12929-017-0362-8. PMID: 28822354; PMCID: PMC5563385.
- Lancaster MA, Knoblich JA. Generation of cerebral organoids from human pluripotent stem cells. Nat Protoc. 2014 Oct;9(10):2329-40. doi: 10.1038/nprot.2014.158. Epub 2014 Sep 4. PMID: 25188634; PMCID: PMC4160653.
- Paşca AM, Sloan SA, Clarke LE, Tian Y, Makinson CD, Huber N, Kim CH, Park JY, O’Rourke NA, Nguyen KD, Smith SJ, Huguenard JR, Geschwind DH, Barres BA, Paşca SP. Functional cortical neurons and astrocytes from human pluripotent stem cells in 3D culture. Nat Methods. 2015 Jul;12(7):671-8. doi: 10.1038/nmeth.3415. Epub 2015 May 25. PMID: 26005811; PMCID: PMC4489980.
- Lancaster MA, Renner M, Martin CA, Wenzel D, Bicknell LS, Hurles ME, Homfray T, Penninger JM, Jackson AP, Knoblich JA. Cerebral organoids model human brain development and microcephaly. Nature. 2013 Sep 19;501(7467):373-9. doi: 10.1038/nature12517. Epub 2013 Aug 28. PMID: 23995685; PMCID: PMC3817409.
- Tao Y, Zhang SC. Neural Subtype Specification from Human Pluripotent Stem Cells. Cell Stem Cell. 2016 Nov 3;19(5):573-586. doi: 10.1016/j.stem.2016.10.015. PMID: 27814479; PMCID: PMC5127287.
- Mungenast AE, Siegert S, Tsai LH. Modeling Alzheimer’s disease with human induced pluripotent stem (iPS) cells. Mol Cell Neurosci. 2016 Jun;73:13-31. doi: 10.1016/j.mcn.2015.11.010. Epub 2015 Dec 4. PMID: 26657644; PMCID: PMC5930170.
- Sances S, Bruijn LI, Chandran S, Eggan K, Ho R, Klim JR, Livesey MR, Lowry E, Macklis JD, Rushton D, Sadegh C, Sareen D, Wichterle H, Zhang SC, Svendsen CN. Modeling ALS with motor neurons derived from human induced pluripotent stem cells. Nat Neurosci. 2016 Apr;19(4):542-53. doi: 10.1038/nn.4273. PMID: 27021939; PMCID: PMC5015775.
- Zhang H, Tian L, Shen M, Tu C, Wu H, Gu M, Paik DT, Wu JC. Generation of Quiescent Cardiac Fibroblasts From Human Induced Pluripotent Stem Cells for In Vitro Modeling of Cardiac Fibrosis. Circ Res. 2019 Aug 16;125(5):552-566. doi: 10.1161/CIRCRESAHA.119.315491. Epub 2019 Jul 10. PMID: 31288631; PMCID: PMC6768436.
- Vijayakumar N, Op de Macks Z, Shirtcliff EA, Pfeifer JH. Puberty and the human brain: Insights into adolescent development. Neurosci Biobehav Rev. 2018 Sep;92:417-436. doi: 10.1016/j.neubiorev.2018.06.004. Epub 2018 Jul 1. PMID: 29972766; PMCID: PMC6234123.
- Martinez-Morga M, Martinez S. Desarrollo y plasticidad del cerebro [Brain development and plasticity]. Rev Neurol. 2016;62 Suppl 1:S3-8. Spanish. PMID: 26922956.
- Stiles J, Jernigan TL. The basics of brain development. Neuropsychol Rev. 2010 Dec;20(4):327-48. doi: 10.1007/s11065-010-9148-4. Epub 2010 Nov 3. PMID: 21042938; PMCID: PMC2989000.
- Dubois J, Dehaene-Lambertz G, Kulikova S, Poupon C, Hüppi PS, Hertz-Pannier L. The early development of brain white matter: a review of imaging studies in fetuses, newborns and infants. Neuroscience. 2014 Sep 12;276:48-71. doi: 10.1016/j.neuroscience.2013.12.044. Epub 2013 Dec 28. PMID: 24378955.
- Semple BD, Blomgren K, Gimlin K, Ferriero DM, Noble-Haeusslein LJ. Brain development in rodents and humans: Identifying benchmarks of maturation and vulnerability to injury across species. Prog Neurobiol. 2013 Jul-Aug;106-107:1-16. doi: 10.1016/j.pneurobio.2013.04.001. Epub 2013 Apr 11. PMID: 23583307; PMCID: PMC3737272.
- Santocchi E, Guiducci L, Fulceri F, Billeci L, Buzzigoli E, Apicella F, Calderoni S, Grossi E, Morales MA, Muratori F. Gut to brain interaction in Autism Spectrum Disorders: a randomized controlled trial on the role of probiotics on clinical, biochemical and neurophysiological parameters. BMC Psychiatry. 2016 Jun 4;16:183. doi: 10.1186/s12888-016-0887-5. PMID: 27260271; PMCID: PMC4893248.
- Lowe VJ, Wiste HJ, Senjem ML, Weigand SD, Therneau TM, Boeve BF, Josephs KA, Fang P, Pandey MK, Murray ME, Kantarci K, Jones DT, Vemuri P, Graff-Radford J, Schwarz CG, Machulda MM, Mielke MM, Roberts RO, Knopman DS, Petersen RC, Jack CR Jr. Widespread brain tau and its association with ageing, Braak stage and Alzheimer’s dementia. Brain. 2018 Jan 1;141(1):271-287. doi: 10.1093/brain/awx320. PMID: 29228201; PMCID: PMC5837250.
- Smith AD, Smith SM, de Jager CA, Whitbread P, Johnston C, Agacinski G, Oulhaj A, Bradley KM, Jacoby R, Refsum H. Homocysteine-lowering by B vitamins slows the rate of accelerated brain atrophy in mild cognitive impairment: a randomized controlled trial. PLoS One. 2010 Sep 8;5(9):e12244. doi: 10.1371/journal.pone.0012244. PMID: 20838622; PMCID: PMC2935890.
- Lin CH, Chiu SI, Chen TF, Jang JR, Chiu MJ. Classifications of Neurodegenerative Disorders Using a Multiplex Blood Biomarkers-Based Machine Learning Model. Int J Mol Sci. 2020 Sep 21;21(18):6914. doi: 10.3390/ijms21186914. PMID: 32967146; PMCID: PMC7555120.
- Toma K, Hanashima C. Switching modes in corticogenesis: mechanisms of neuronal subtype transitions and integration in the cerebral cortex. Front Neurosci. 2015 Aug 11;9:274. doi: 10.3389/fnins.2015.00274. PMID: 26321900; PMCID: PMC4531338.
- Aprea J, Calegari F. Long non-coding RNAs in corticogenesis: deciphering the non-coding code of the brain. EMBO J. 2015 Dec 2;34(23):2865-84. doi: 10.15252/embj.201592655. Epub 2015 Oct 29. PMID: 26516210; PMCID: PMC4687686.
- Ishii K, Kubo KI, Nakajima K. Reelin and Neuropsychiatric Disorders. Front Cell Neurosci. 2016 Oct 18;10:229. doi: 10.3389/fncel.2016.00229. PMID: 27803648; PMCID: PMC5067484.
- Subramanian L, Calcagnotto ME, Paredes MF. Cortical Malformations: Lessons in Human Brain Development. Front Cell Neurosci. 2020 Jan 24;13:576. doi: 10.3389/fncel.2019.00576. PMID: 32038172; PMCID: PMC6993122.
- Trevino AE, Sinnott-Armstrong N, Andersen J, Yoon SJ, Huber N, Pritchard JK, Chang HY, Greenleaf WJ, Pașca SP. Chromatin accessibility dynamics in a model of human forebrain development. Science. 2020 Jan 24;367(6476):eaay1645. doi: 10.1126/science.aay1645. PMID: 31974223; PMCID: PMC7313757.
About the author
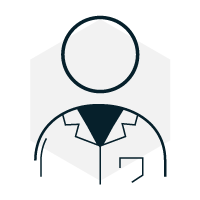
Harsheel Dhruva
Harsheel is currently a Senior at Irvington High School in Fremont, California. Growing up in the Bay Area, he is very interested in the natural sciences and the potential of discovery from advancing technology. He is pursuing studies in Neuroscience and Biotechnology in hope of pioneering his own research in the future and create technology to improve the human condition. Outside of his study, Harsheel loves completing challenging physical activity or listening to music whenever he gets the chance, and is always happy to read something new.