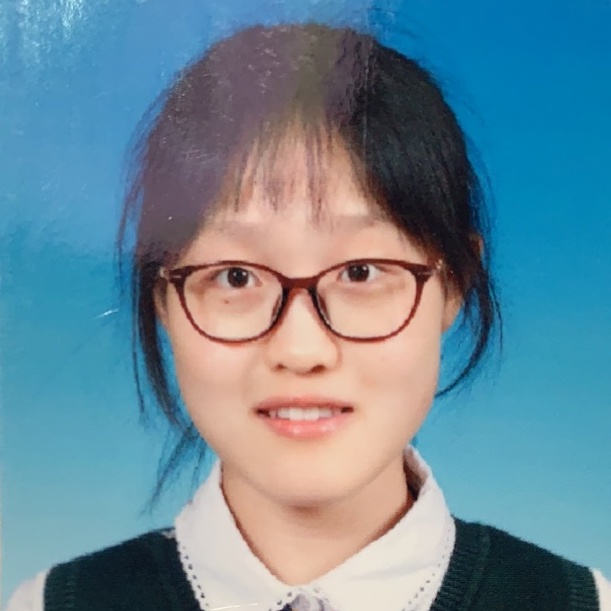
Author: Ashley Qian
Mentor: Fabiola Munarin
Wuxi Big Bridge Academy
October 1, 2021
Abstract
Sleep has been the center of discussion for scientists over nearly the past century, with compelling evidence that show sleep influences memory consolidation. Nervous systems have specific structures related to distinctive functions, which serves to transform short-term memories into long-term memories, with synapses playing a crucial role. Tests on electrophysiology markers of synaptic efficacy adds proof to the synaptic homeostasis (SHY) hypothesis. During sleep, spontaneous activity renormalizes net synaptic strength and restores cellular homeostasis. Sleep stages and rhythms correspond to 5 types of brain waves respectively, and there are interplays between sleep rhythms and memory consolidation. Explicit memories (associated with neocortex and amygdala), reported by traditional studies, are strengthened during SWS sleep, whereas implicit memory (associated with basal ganglia and cerebellum) mechanisms have been also demonstrated in recent research to be recruited during NREM sleep periods. Experiments have provided evidence that show how individuals reproduce knowledge they learned after retention of several hours after intervals of sleep or wakefulness respectively. Data analysis show that sleep promotes memory consolidation consistently; effects are extremely significant in the situation with declarative memories (syllables). Further research done recently have also come up with the result of brain reactivation during sleep.
1. Introduction
The basics of memory formation involve three stages: encoding, storage, and retrieval. Encoding represents the transformation of sensory inputs into short-term and long-term memories, storage is defined as to maintaining the encoded sensory information over time, whereas retrieval refers to how people get access to the actual memories stored in their brain. Different mechanisms, as well as different brain areas, are triggered when humans are forming and storing memories. For example, short-term memory involves mainly the prefrontal cortex, while long term memory involves the hippocampus, neocortex and amygdala (explicit memory) or the basal ganglia and cerebellum (implicit memory). Explicit memories, which are episodic events and semantic information, together with implicit memories, which involve motor memories, make up long-term memories.
Memory consolidation is the rehearsal of earlier memories resulting in the increasing number of synapses, swelling amount of neurotransmitter released, and enlarging number of receptors on postsynaptic membrane. By consolidation, short-term memories can develop into long-term memories. Memory consolidation is rather significant. It is reported that students on average are able to grasp only 25% of the lecture they are listening to even carefully. The left 75% requires the mechanism of memory consolidation to be in the storehouse where it should be. Hence, teachers are supposed to assign homework and give feedbacks. This can be named as early consolidation practice which is later strengthened by quizzes and examinations.
Over the last decades, scientists have gained compelling evidence that sleep enhances the formation of long-term memories. Traditional theories proposed a passive role for sleep supporting memories by sheltering them from external stimuli, however recent research have uncovered an active role for sleep during memory consolidation. Traditional studies emphasized the role of rapid-eye-movement (REM) sleep, present papers have revealed that slow-wave-sleep (SWS) is also significant (Rasch B, Born J. 2013). Initially, scientists emphasize that sleeping provides shelter for the brain’s memory storage from external stimuli or interferences; yet later researchers put forward that sleep actively involves in system consolidation of memories. In this review, we will summarize about the correlations of sleep and memory consolidation.
- Neuron physiology
- Neuronal structures and functions
Neurons (also called nerve cells or neurones) are the fundamental units of the brain and nervous system. They are responsible for receiving input from external world, sending commands to the muscles, and relaying or integrating electrical signals. Most of a neuron’s organelles are in the cell body, with the largest karyoplasmic ratio of approximately 1:1 among all the other cell types.
A useful model is to consider the structure of a neuron as a tree, consisting of three major parts: an axon (tree root), dendrites (tree branches), and a cell body (tree trunk). Long and slender, axons are projections of nerve cells that conducts action potential away from the cell body. The primary function of axons is to transmit signals to target cells. Axons fall into two categories: myelinated and unmyelinated. Myelinated axons are surrounded by segments of a fatty layer of insulating glial cells, namely Schwann cells (in the CNS) and oligodendrocytes (in the PNS). Vacant space in myelin sheath is known as nodes of Ranvier, where action potentials jump along. Due to their lengths, axons can be divided into branches of telodendrions, whose ends are called axon terminals. Axon terminals, as pre-synaptic axons, contain vesicles of neurotransmitters for the relay of electrical signals in the synapses. The post-synaptic dendrites can be easily distinguished by its similar appearances to lushy but short branches. Dendrites receives signals from axons and carries the action potential towards the soma. Dendrite spines are small protrusions that increase the receptive properties of dendrites to isolate signal specificity. Implication of dendrite spines can be seen in long-term potentiation. In other words, the ability of dendritic development plays a crucial role in memory formation. The cell body, the soma, holds nucleus responsible for production of proteins.
The site in the nervous system where signals are relayed is called synapses. As signals can be described as chemical and electrical, so as synapses. In chemical synapses, the electrical signals must first trigger the release of neurotransmitters and next the second messenger or be again converted into electrical signals. While in electrical synapses, currents past through gap junctions directly by inducing voltage changes in the postsynaptic cells. Without the multiple steps used in chemical synapses, electrical ones are special for their rapidity (Cirelli C. 2013).
When not functioning, a neuron has a rest potential of 60-70 mV across its membrane, with the interior more negatively-charged. Opening or closing ion channels in the membrane lead to changes in the potentials. Hyperpolarization is when the membrane potential becomes more negative, with depolarization the opposite. Opening of channels that let potassium cations out or chlorine anions in may results in hyperpolarization. On the other hand, opening of channels that let sodium cations in will cause depolarization. When multiple depolarizing inputs happen at the same time, a large enough depolarization may ultimately lead to an action potential. An action potential is an all-or-none event, which means the stimulus must thrust the threshold of negative 55 mV. At this threshold, sodium cations influxes to increase the potential up to 40mV. Next, influxes stop and potassium cations rush out, causing a rapid fall towards normal resting state. Yet the potassium cations keep flowing out, resulting in a period of undershoot, where even larger potentials are needed to induce another action potential. Eventually, potassium outfluxes stop and the potential returns to -60 to -70 mV, ready for the next cycle. (Reece, J. B., et al, 2011)
1.12 Electrophysiology of synapses during wakefulness and sleep
Electrophysiological markers of synaptic efficacy have been tested in recent decades. The slope of the early response evoked by electrical stimulation delivered within a cell is a classical electrophysiological measure of synaptic strength, with a steeper slope indicating higher synaptic efficiency. A recent study utilized transcranial magnetic stimulation to evoke a response in humans’ frontal cortex. Results have shown that the slope of early responses increased gradually over the period of 18 hours of consisting awareness, and then recover to basic levels after one night of sleep (Cirelli, Chiara,2013). The explanation for such phenomenon has been proposed. The synaptic homeostasis hypothesis (SHY) proposes that sleep is the price the brain pays for plasticity. During a waking episode, learning statistical regularities about the current environment requires strengthening connections throughout the brain. This increases cellular needs for energy and supplies, decreases signal-to-noise ratios, and saturates learning. During sleep, spontaneous activity renormalizes net synaptic strength and restores cellular homeostasis. Activity-dependent down-selection of synapses can also explain the benefits of sleep on memory acquisition, consolidation, and integration (Tononi, G, et al, 2014).
- Correlations between sleep and memory consolidation
- Sleep stages and brain waves
As measured by eletroencephalography (EEG), the brain’s electrical activity is determined by the presence of brain waves, which fall into 5 categories: Gamma, Beta, Alpha, Theta and Delta waves. Gamma waves, are small-amplitude high-frequency (25-140 Hz) ones related to hyper brain activity, such as problem solving and high-concentration tasks. Beta waves (14-25 HZ) possess both lower amplitude and frequency traits and are generated when the brain is busily engaged in activities and conversation. Alpha waves (7.5-13 Hz); Theta waves (3.5-7.5 Hz) and Delta waves (3Hz or below) are all associated with rest, sleep and dreaming activities.
During the approximately 90-minute-long sleep cycle, non-REM sleep first appears and rapid-eye-movement sleep (REM) follows. The sleep cycle has four stages, that are characterized by the presence of different brain waves. Stage 1 represents the transition step between wakefulness and sleep, in which the brain generates Alpha waves. The following stage 2 is where Theta waves, K-Complex and sleep spindle waves alternate as responses and inhibition towards outside stimuli. While progressing to stage 3 and 4 sleep, people are drifting down into deeper sleep, and the brain emits Delta waves. Finally, people fall into stage 5 sleep, or REM sleep, presenting bran waves similar to those of an awake person. In this phase, dreaming occurs and the skeletal muscles experience atonia, and are unable to move. Cycles of slow wave and REM sleep alternate at night, with the slow wave sleep becoming less deep and the REM periods more prolonged until awakening.
2. Consolidation during SWS and REM
Memory consolidation happens during slow wave sleep and REM phase. Slow wave sleep (SWS) periods appear to strengthen explicit memories evidenced by the observations on increased SWS after intensive episodic learning (Acosta MT, 2019). Recent studies on implicit memories during sleep have also demonstrated the recruitment of implicit learning mechanisms during NREM sleep, suggesting that memory consolidation may be optimized when taking into account sleep stages and sleep rhythms (Born J, et al, 2012).
During slow wave sleep, the experienced episodes are stored temporarily in the hippocampus and are then redistributed in a long-term store in the neocortex. The waves generated in this process are hippocampal ripples, thalamocortical spindles and slow waves (delta waves). Originating in the medial temporal lobe, hippocampal ripples have a frequency of 100-300 Hz. Thalamocortical spindles are typical waves for stage 2 and SWS sleep, with a frequency of 10-15 Hz (Gage et al, 2018). Spindles reaching the neocortex likely act to prime respective neuronal networks, e.g., by stimulating Ca2+ influx for subsequent synaptic plastic processes. Thus, memory information carried in single troughs of spindle oscillations maybe particularly effective in changing synaptic connections underlying the long-term storage of the information in the respective neocortical networks (Born et al, 2012). The third key SWS oscillation are the slow waves (0.5 – 4Hz). Slow waves are considered to reflect the brain’s up-and-down states, which is critical for memory redistribution, i.e., the encoding of information. The more information is encoded during wakefulness the higher amplitude of the slow oscillations is generated over respective cortical areas during succeeding SWS(Schonauer, et al. 2014). A major function of the slow oscillations is that they temporally group neuronal activity into hyperpolarizing down-states during which neurons are globally silent and succeeding depolarizing up-states during which neuronal firing is increased to wake-like levels (Steriade et al. 2006). As previously described, the hippocampus, thalamus, and the cortex combine to form the complex neural symphony that corresponds to a key aspect of human SWS: memory stabilization and consolidation.
3. Effects of sleep on memory consolidation
3.1 Performance of individual subjects on the LGT-3 total score
Primary research has found that sleep consistently promotes overall declarative memory consolidation. Forty healthy adults aged 18–30 y, and 17 healthy adults aged 24–55 y with extensive meditation experience participated in the 3 experiments (Schonauer et al, 2014). In Experiment 1, subjects learned Turkish in the afternoon followed by a 2-h sleep, and after a 3-h retention they were tested. Results shown people performing far better after sleep than after wakefulness. There was only one exception that shown better results after an interval of wakefulness. In Experiment 2, volunteers acquired knowledge in the morning, after which they took a 2-h sleep and were tested after 8-h retention. Statistical analysis comported with results from experiment 1, with exception of 2 of 18 subjects (Schonauer et al, 2014). In Experiment 3, procedures were same with Experiment 1 expect for the three states (sleep/active awake/meditation) subjects were kept respectively to compare. Subjects performed better on the test when there were only 3 h between learning and testing than when encoding and testing were spaced 8 h apart. There was still one exception out of 18 subjects. Conclusions were drawn: sleep does improve the consolidation of declarative memories while such effect is reserved only to sleep, and does not happen in the case of wakefulness.
3.2 Significant improvements on syllable memories
Two OS, L. R. Hodell and J. S. McGraw served as subjects and were required to learn meaningless syllables in series of ten by reading aloud, with exposure at the rate of 0.7 sec (Jenkins et al. 2021). The series were repeated by the subjects until they completely remembered the syllables. The experiment was carried out at night, when subjects go to bed after learning. They were separately waked by Ebbinghaus, the scientist, to give reproduction of the syllables. Analysis of data shown superiority of the reproductions after intervals of sleep. This superiority became more and more prominent as the length of the intervals elongated (Jenkins et al. 2021).
3.3 Improve memory by protection or reactivation of the brain during sleep
Sleep after learning leads to superior recall of syllables after the 1-, 2-, 4-, and 8-h retention interval, compared with wake intervals of the same length. Two subjects (H. and Mc.) participated in this classic study by Jenkins and Dallenbach. For each data point, each participant completed 6–8 trials, with the different retention intervals performed in random order (Rash et al. 2013). A time dependency of the effects of sleep on memory formation is indicated by studies showing stronger effects for sleep occurring shortly after learning than for sleep at a later time. For example, sleep occurring within 3-h after learning vocabulary was more beneficial than sleep delayed by more than 10 h. Furthermore, recall of word pairs after 24-h was better when sleep occurred immediately after learning than after a day of wakefulness.Whereas initially it was commonly assumed that sleep improves memory in a passive manner, by protecting it from being overwritten by interfering external stimulus inputs, the current theorizing assumes an active consolidation of memories that is specifically established during sleep, and basically originates from the reactivation of newly encoded memory representations (Rash et al. 2013). Maquet and colleagues (Maquet et al. 2000) led first experiments to mark out the activation of brain in learning using PET. Although there have been plenty of current studies on the evidence of reactivation of the brain using PET, fMRI, and EEG, higher resolution images will be needed to determine when and where reactivations occur.
Conclusions
There’s no deny that sleep is fundamental for memory formation, as proved by studies down recently, which showcased significant enhancements in implicit memories and episodic learning in SWS and REM respectively, syllable learnings being the most evident. However, there’s still great needs for higher resolution images to further decipher the exact mechanisms of latest discovered reactivations during sleeping.
References
- Schonauer, Monika et al. “Exploring the effect of sleep and reduced interference on different forms of declarative memory.” Sleep (2014) vol. 37,12 1995-2007.
- Born, Jan, and Ines Wilhelm. “System consolidation of memory during sleep.” Psychological research vol. 76,2 (2012): 192-203.
- Diekelmann, S., Born, J. The memory function of sleep. Nat Rev Neuroscience 11, 114–126 (2010).
- Born J, Wilhelm I. System consolidation of memory during sleep. Psychol Res. 2012 Mar;76(2):192-203.
- Rasch B, Born J. About sleep’s role in memory. Physiology Rev. 2013 Apr;93(2):681-766.
- Reece, J. B., Urry, L. A., Cain, M. L., Wasserman, S. A., Minorsky, P. V., and Jackson, R. B. (2011). Neurons, synapses, and signaling. In Campbell biology (10th ed., pp. 1061-1078). San Francisco, CA: Pearson.
- Cirelli, Chiara. “Sleep and synaptic changes.” Current opinion in neurobiology vol. 23,5 (2013): 841-6.
- Cirelli C. Sleep and synaptic changes. Current opinion in neurobiology. 2013 Oct;23(5):841-6.
- Tononi, Giulio, and Chiara Cirelli. “Sleep and the price of plasticity: from synaptic and cellular homeostasis to memory consolidation and integration.” Neuron vol. 81,1 (2014): 12-34.
- Acosta MT. Sueño, memorial appendixes [Sleep, memory and learning]. Medicina (B Aires). 2019;79 Suppl 3:29-32.
- Donlea JM. Roles for sleep in memory: insights from the fly. Current opinion in neurobiology. 2019 Feb; 54:120-126.
- Pace-Schott EF, Germain A, Milad MR. Effects of sleep on memory for conditioned fear and fear extinction. Psychol Bull. 2015 Jul;141(4):835-57.
- Diering GH, Nirujogi RS, Roth RH, Worley PF, Pandey A, Huganir RL. Homer1a drives homeostatic scaling-down of excitatory synapses during sleep. Science. 2017 Feb 3;355(6324):511-515.
- Acsady L, Harris KD. Synaptic scaling in sleep. Science. 2017 Feb 3;355(6324):457.
- Rasch Bjorn, Born Jan. “About Sleep’s Role in Memory.” Physiological Reviews vol. 93, No. 2. 2013 Apr.
- Jenkins, John G., and Karl M. Dallenbach. “Obliviscence during Sleep and Waking.” The American Journal of Psychology, vol. 35, no. 4, 1924, pp. 605–612.
- Azarfar A, Calcini N, Huang C, Zeldenrust F, Celikel T. Neural coding: A single neuron’s perspective. Neurosci Biobehav Rev. 2018 Nov;94:238-247.
- Reuveni I, Barkai E. Tune it in: mechanisms and computational significance of neuron-autonomous plasticity. J Neurophysiol. 2018 Oct 1;120(4):1781-1795.
- Verkhratsky A, Ho MS, Parpura V. Evolution of Neuroglia. Adv Exp Med Biol. 2019;1175:15-44.
- Stiefel KM, Ermentrout GB. Neurons as oscillators. J Neurophysiol. 2016 Dec 1;116(6):2950-2960.
- Berry KP, Nedivi E. Spine Dynamics: Are They All the Same? Neuron. 2017 Sep 27;96(1):43-55.
- Choquet D, Triller A. The dynamic synapse. Neuron. 2013 Oct 30;80(3):691-703.
- Fabricant A, Iwata GZ, Scherzer S, Bougas L, Rolfs K, Jodko-Władzińska A, Voigt J, Hedrich R, Budker D. Action potentials induce biomagnetic fields in carnivorous Venus flytrap plants. Sci Rep. 2021 Jan 14;11(1):1438.
- Fields RD. Oligodendrocytes changing the rules: action potentials in glia and oligodendrocytes controlling action potentials. Neuroscientist. 2008 Dec;14(6):540-3.
- Xie C, Lin Z, Hanson L, Cui Y, Cui B. Intracellular recording of action potentials by nanopillar electroporation. Nat Nanotechnol. 2012 Feb 12;7(3):185-90. doi:
- Ackermann S, Rasch B. Differential effects of non-REM and REM sleep on memory consolidation? Curr Neurol Neurosci Rep. 2014 Feb;14(2):430.
- Gottesmann C. Detection of seven sleep-waking stages in the rat. Neurosci Biobehav Rev. 1992 Spring;16(1):31-8.
- Rotenberg VS. Sleep and memory. I: The influence of different sleep stages on memory. Neurosci Biobehav Rev. 1992 Winter;16(4):497-502.
- Brancaccio A, Tabarelli D, Bigica M, Baldauf D. Cortical source localization of sleep-stage specific oscillatory activity. Sci Rep. 2020 Apr 24;10(1):6976.
- Biddle C, Oaster TR. The nature of sleep. AANA J. 1990 Feb;58(1):36-44.
About the author
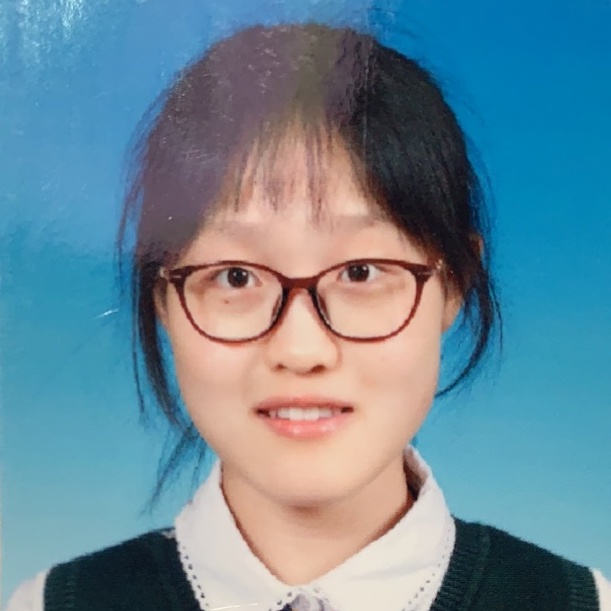
Ashley Qian
Ashley is currently a Junior at the Wuxi Big Bridge Academy.